Breakthrough in Ultrafast Tunable Lasers with Lithium Niobate Integrated Photonics
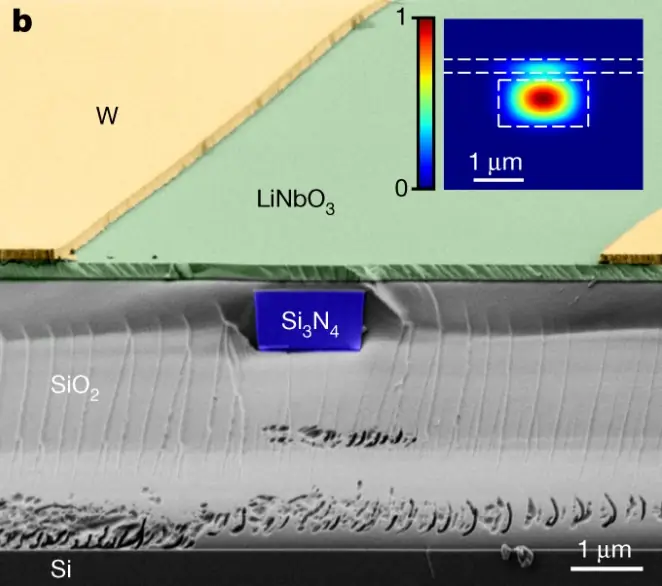
In a groundbreaking development, scientists have successfully demonstrated the capabilities of ultrafast tunable lasers using lithium niobate (LiNbO3) integrated photonics. These lasers showcase narrow linewidth while maintaining extreme frequency agility, allowing for tuning rates at petahertz per second. This innovation has broad implications for applications such as frequency-modulated continuous-wave (FMCW) light detection and ranging (LiDAR), optical coherence tomography, frequency metrology, and trace-gas spectroscopy.
Lithium Niobate’s Role in Electro-Optic Devices
Lithium niobate is a popular material for electro-optic devices, having been in use for several decades. It features a wide transparency window from ultraviolet to mid-infrared wavelengths and a large Pockels coefficient of 32 pm V−1. This allows for efficient, low-voltage, and high-speed modulation. However, previous attempts to create integrated lasers with LiNbO3-based photonic circuits have not achieved the full potential of frequency-agile and narrow-linewidth integrated lasers.
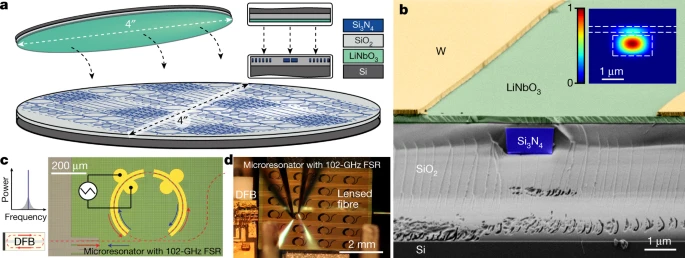
Innovative Hybrid Si3N4–LiNbO3 Platform
In this new approach, researchers combined the best properties of ultralow-loss silicon nitride (Si3N4) photonic waveguides with thin-film lithium niobate by wafer-scale bonding. The heterogeneously integrated platform uses a Si3N4–LiNbO3 chip that is butt-coupled to an indium phosphide (InP) distributed feedback (DFB) diode laser. The Si3N4 photonic integrated circuits feature tight optical confinement, ultralow propagation loss (<2 dB m−1), low thermal absorption heating, high-power handling, and can be manufactured at the wafer scale with high yield. Additionally, the Si3N4 platform is known for its low gain from Raman and Brillouin nonlinearities and radiation hardness.
This hybrid Si3N4–LiNbO3 platform results in high-Q microresonators with a median intrinsic cavity linewidth of 44 MHz, providing a near-unity yield of bonded devices and a low insertion loss of 3.9 dB per facet. The platform also does not exhibit bend-induced mode mixing due to birefringence, which is common for LiNbO3 ridge waveguides. By integrating the unique properties of both materials into a single heterogeneous platform, researchers achieved laser self-injection locking with two orders of magnitude of laser frequency noise reduction and a petahertz-per-second frequency tuning rate.
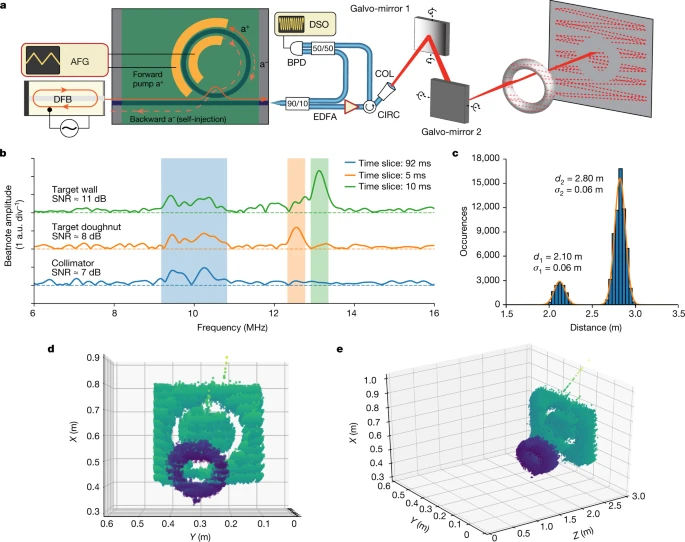
Proof-of-Concept and Future Implications
As a proof-of-concept, the team conducted a coherent optical ranging (FMCW LiDAR) experiment using the hybrid integrated laser. This development opens up new possibilities for utilizing the individual advantages of thin-film LiNbO3 and Si3N4, combining precise lithographic control, mature manufacturing, and ultralow loss in a single platform.
In summary, this breakthrough in ultrafast tunable lasers using lithium niobate integrated photonics has significant potential for a wide range of applications. The combination of silicon nitride and lithium niobate in a single platform paves the way for further advances in integrated laser technology and its various applications.